STEM CELL BIOENGINEERING
(August 2004)
Stem cells have generated more excitement, scrutiny and controversy than any other area of recent scientific study. The first stem cells, which were discovered half a century ago, were isolated from blood. Now, scientists around the globe are researching various types of stem cells for their potential to regenerate lost tissue and revolutionize medicine.
Embryonic stem (ES) cells are derived from the embryo when it exists as a blastocyst. They have the ability to develop into all the different cell types found in the body. Actually, when a sperm fertilizes an egg, the resulting single cell begins to divide and multiply at a rate much faster than that observed in somatic cells. Scientists refer to these cells as totipotent stem cells (Figure 1). These primordial embryonic cells have the potential to grow into a complete mammal. Within days of fertilization, these new and dividing cells form a hollow sphere, called a blastocyst. Stem cells arising in the inner mass of the blastocyst are called the ES cells. The ES cells are considered pluripotent – they can divide indefinitely and blossom into all the various tissue types of the human body, but they have the lost the totipotent ability to grow into a separate being. After roughly 14 days, ES cells divide to give rise to what will eventually develop into the spine. At this stage, the stem cells within the embryo are considered multipotent. These stem cells can grow into some tissues, but not all tissues. Those destined to become bone or blood, for example, may not be able to form stomach or skin.
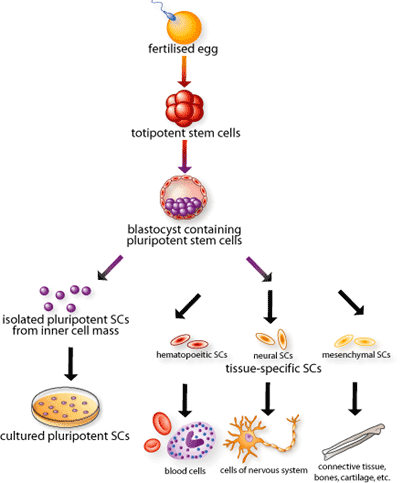
By definition, stem cells have two important characteristics that distinguish them from other types of cells. First, they are unspecialized cells that renew themselves for long periods of time through cell division of at least one daughter cell. Secondly, under certain physiological or experimental conditions, they can be induced to differentiate. This means that they can divide into cells with special functions, such as the beating cells of the heart muscle or the insulin-producing cells of the pancreas.
Discovery of ES Cells
The work that laid the foundation for ES cell discovery was the study of teratocarcinomas, complex tumors containing a mix of specialized cell types as well as a population of unspecialized cells. These unspecialized cells are called embryonal carcinoma (EC) cells. The latter were shown to be pluripotent and could give rise to various cell types both in vitro and in vivo. It was therefore natural to consider using these cells for therapeutic purposes. However, EC cells never seemed ideal for this purpose because they had an abnormal number of chromosomes and originated from tumors. Careful study of the induction of teratocarcinomas in experimental animals, as well as an understanding of the biology of EC cells and early embryos, led scientists to the discovery of ES cells in the early 1980s. The demonstration that ES cells contained the normal number of chromosomes and were truly pluripotential has influenced many scientific disciplines.
Biological role and properties of stem cells
Stem cells differ from other kinds of cells in the body. All stem cells, regardless of their source, have three general properties: they are capable of dividing and renewing themselves for long periods; they are unspecialized; they can choose to become one of the many different types of cells present in the body based on signals from their environments.
Finding the answers to two fundamental questions about stem cells that relate to their long-term self-renewal is crucial to our ability to successfully grow these cells in laboratory and in turn use them for various tissue engineering and cellular therapies. The first question deals with why embryonic stem cells can proliferate for extended periods of time in the laboratory without adopting a specialized fate, while most adult stem cells cannot. The second issue addresses which factors in living organisms normally regulate stem cell self-renewal and differentiation. Discovering the answers to these questions may make it possible to understand how cell proliferation is regulated during normal embryonic development or during the abnormal cell division that ultimately leads to cancer.
Stem cells are unspecialized
One of the fundamental properties of stem cells is that they do not perform specialized functions. A stem cell cannot pump blood through the body (like a heart muscle cell) or supply oxygen to the cells of the body (like a red blood cell). However, unspecialized stem cells can give rise to specialized cells including heart muscle cells, blood cells, or nerve cells. They do this by coordinating their gene expression in an elaborate and complex pattern spanning many generations of cells.
Stem cells are self- renewing
Most specialized cells in our body (such as muscle cells, blood cells, or nerve cells) do not replicate themselves. The supply of these cells is maintained by stem cells, which replicate many times and then differentiate into the specialized cells that are needed. In this way, cells are continuously replenished as they die. This is called homeostasis.
When cells replicate themselves many times over it is called proliferation. A starting population of stem cells that proliferates for many months in the laboratory can yield hundreds of millions of cells. If the resulting cells are identical (in terms of being unspecialized themselves and capable of generating specialized cells) to the parent stem cells, the ES cells are said to be capable of long-term self-renewal. Scientists are greatly interested in determining specific factors and conditions that allow stem cells to remain unspecialized in the laboratory for long periods of time.
Stem cells can give rise to specialized cells
Differentiation is the process whereby stem cells give rise to specialized cells. Scientists are just beginning to understand the signals that trigger stem cell differentiation. These signals include chemicals secreted by other cells, physical contact with neighboring cells, and contact with molecules in the microenvironment.
Can specific sets of signals be identified as responsible for the promotion of differentiation into specific cell types? Answering this question is critical in order to learn how to maintain pools of stem cells. The development of stem cell-based technologies will depend on our ability to reproducibly drive the differentiation of stem cells into specific tissue lineages. This issue is of particular importance for the development of ES cell-based technologies, where the differentiation of certain subsets of cells and tissues is uncommon. The generation of ES cell-derived heart muscle cells is one example where significant improvements in tissue-specific differentiation are needed. Under default mechanisms, only approximately 1% of ES cells form cardiac tissue.
Growing embryonic stem cells in the laboratory
The process of growing cells in the laboratory is known as cell culture. Mouse (or human) embryonic stem cells are isolated by transferring the inner cell mass of the blastocyst into a plastic laboratory culture dish containing medium, which provides nutrients and necessary growth factors. The cells divide and spread over the surface of the dish. In the case of human ES cells, the inner surface of the culture dish is typically coated with mouse embryonic skin cells, which are non-dividing and secrete a host of growth factors. This is known as a feeder layer and is necessary for the prevention of ES cell differentiation. The feeder layer provides a sticky surface to which the inner cell mass cells can attach. Mouse ES cells, however, can be maintained in culture without the need of a feeder layer. In this case, the culture medium is supplemented with high concentrations of a protein known as leukemia inhibitory factor (LIF), which prevents differentiation.
Over the next several days, the cells of the inner cell mass grow and divide and spread on the culture dish. When the cell concentration is high enough, they are removed gently and plated into several fresh culture dishes. This process of replating the cells is repeated for many months, and is called subculturing. After six months or more, the original 20 to 30 cells of the inner cell mass yield many millions of embryonic stem cells. When embryonic stem cells have proliferated in cell culture for six or more months without differentiating, are pluripotent, and appear to be genetically normal, they are referred to as an ES cell line. Once ES cell lines are established, batches of them can be frozen and kept for further experiments or even shared with other laboratories for further culture and experimentation.
ES Cell Differentiation
As long as mouse ES cells in culture are grown in the presence of LIF or a feeder layer, they can remain undifferentiated (unspecialized). If LIF is removed from the culture medium, cells will begin to clump together to form 3-dimensional structures called embryoid bodies (EBs). The cells then begin to differentiate spontaneously. Allowing spontaneous differentiation is a good way to determine if a culture of embryonic stem cells is healthy. It is not an efficient way to produce cultures of a specific cell type. In order to generate a large number of cells of a specific type, scientists use the process of directed differentiation. This is done by changing the chemical composition of the culture medium, altering the surface of the culture dish, or modifying the cells by inserting specific genes.
Adult Stem Cells
Many adult tissues (such as the bone marrow, brain and gut) contain stem cells. Like ES cells, adult stem cells can make identical copies of themselves for long periods of time (self-renewal). At the same time, they can give rise to mature cell types that have characteristic shapes and specialized functions. Stem cells typically generate an intermediate cell type(s) before they achieve their fully differentiated state. The intermediate cell is called a progenitor cell. Progenitor cells are partly differentiated cells in the sense that they are committed to a particular cell lineage and, upon division, and give rise to differentiated cells. Of all the adult stem cells identified thus far, Hematopoeitic stem cells (HSCs) are the best characterized.
Stem cell plasticity
Until recently, adult stem cells were considered to be irreversibly committed to specific lineages of differentiation. HSCs, for example, normally give rise to all types of blood cells such as red blood cells, white blood cells and platelets. It was previously believed that HSCs could not give rise to any cells of a different tissue. However, a number of experiments over the last several years have raised the possibility that stem cells from one tissue may be able to give rise to cell types of a completely different tissue. This phenomenon is known as ‘stem cell plasticity’. Examples of such plasticity include bone marrow stem cells becoming neurons, or pancreatic islet cells that are capable of producing insulin. Exploring the possibility of using adult stem cells for cellular therapies has recently become an active area of research. The apparent plasticity of adult stem cells has forced scientists to reconsider many fundamental concepts of stem cell biology. Many new questions arise from these studies. For instance, are the recently characterized stem cells typical tissue-specific stem cells? Might they be a sub-population of stem cells with a developmental potential closer to that of embryonic stem cells? There is a possibility that stem cell plasticity results from in vitro manipulations and does not reflect normal behavior in vivo. Regardless, this phenomenon has important clinical implications.
Potential Therapeutic Applications of Stem Cells
The ultimate objective of stem cell bioengineering is to be able to understand and possibly control stem cell differentiation and lineage commitment in vitro. If this can be achieved, a multitude of therapeutic applications can be envisioned. One potential application is the generation of different types of neurons for the treatment of Alzheimer’s disease, spinal cord injuries, or Parkinson’s disease. The production of heart muscle cells for heart attack survivors may also be possible. The generation of insulin-secreting pancreatic islet cells for the treatment of type-1 diabetes, and even the generation of hair follicle stem cells for the treatment of certain types of baldness, have been considered. Stem cells could also be useful for a number of tissue engineering applications such as the production of complete organs including livers, kidneys, eyes, hearts, or even parts of the brain. This represents a considerably greater challenge, beyond the generation of specialized cell types, and will require considerable time and effort to develop. Other areas that would benefit from a better understanding and control of stem cell proliferation in vitro are drug testing, cancer research, and fundamental research on embryonic development.
Human ES cells could potentially be used for all of the above applications. Human ES cells are obtained from aborted fetuses or fertilized eggs. This has come under ethical scrutiny since use of these procedures requires serious moral consideration by society. A possible way to circumvent this issue would be to use stem cells isolated from adult tissues. If, as recent studies have suggested, the plasticity of stem cells is greater than previously imagined, then it may be feasible to use adult stem cells as an initial cell population for therapeutic modality. Bone marrow, peripheral blood or umbilical cord blood HSCs could be ideal for such procedures. They are relatively easy to harvest and robust assays exist to quantify HSCs and distinguish them from more differentiated progenitors. Although much remains to be learned about the factors controlling their self-renewal and differentiation, the state of knowledge about HSCs is considerably more advanced than for other stem cell systems.
(Art by Jen Philpott)