FROM DYES TO PEPTIDES: THE EVOLUTION OF ANTIBIOTIC DRUGS
(August 2004)
In the last century, nothing has made a bigger impact on human health than antimicrobial chemotherapy [1]. After 20 years of clinical use, antibiotics have increased the average human life expectancy by ten years while in comparison, curing cancer would only only extend life expectancy by two years [1]. From 1900 to 1990, the average life expectancy of citizens of the U.S. increased a staggering 29 years. In fact, in most of developed countries, mortality due to infectious disease has largely been replaced with mortality due to chronic illnesses such as heart disease, cancer and strokes. Although the decrease in infectious disease can also be attributed to better nutrition, better housing, safer food and water and improved hygiene and sanitation, the major catalyst for the improvement of overall human health in the last century is most likely the advent of antibiotics. [2]
The Search for the Magic Bullet
In the late 1800’s, Paul Elrich, now known as the father of antibiotic therapy [3], was becoming increasingly interested in staining tissues and was particularly fascinated by differential staining of bacterial cells and human tissue cells. He hypothesized that if a toxic dye could be taken up by bacterial cells and not human cells, then bacteria could be selectively killed, thus eradicating infections [3]. This dye would be the “magic bullet” that microbiologists were searching for. Elrich began testing numerous dyes with no luck and so began to chemically alter them little by little. In total, Elrich tested 606 arsenic compounds, and in 1909, compound number 606, arsphenamine (later called salvarsen) was shown to destroy the syphilis-causing pathogen Treponema pallidum, and was successfully used to treat cases of syphilis [3]. At the time syphilis was a fatal and incurable sexually transmitted infection, just as AIDS is today, and so the discovery of an effective treatment had a huge impact on the medical community3. Although arsphenamine was used to treat many syphilitic patients, it was extremely toxic and some patients died from it. Arsphenamine was unfortunately not the selective “magic bullet” that Elrich had been searching for [3]. In spite of this, Elrich eventually received the Nobel Prize in medicine in 1908 for ‘outlining the principles of selective toxicity and for showing preferential eradication of cells by chemicals’. [3]
While Elrich was working with arsphenamine, a German chemical company, IG Farbenindustrie, was putting together a research facility to test dyes against infectious agents [3]. In 1932, Gerhard Domagk tested a red dye, Prontosil, against Streptococcus infections in mice. This dye was able to successfully eradicate the infection and drug testing progressed to human patients. Despite its effectiveness, Prontosil was not largely accepted by the medical community and was subject to intense speculation [3]. It wasn’t until 1936, when President Roosevelt’s son developed severe tonsillitis and as a final measure was treated with Prontosil did the drug achieve real popularity among doctors and scientists. The boy made a complete recovery and the New York Times announced that a “New Control for Infections” had been discovered [3]. Although some American physicians were still unenthusiastic about the drug, people rushed to use it [3].
Pharmaceutical competitors soon began to look for ways to circumvent IG Farbenindustrie’s patent on Prontosil [3]. Eventually French chemists found that the dye’s antibiotic properties were actually due to a breakdown product in the dye that they called sulphanilamide. IG Farbenindustrie’s patent on this compound had long expired and so sulphanilamides were free to be synthesized by anybody who cared to make them, and so Domagk didn’t profit much from his discovery [3]. However, like Elrich, he still achieved fame in 1939 when he was awarded the Nobel Prize. Unfortunately he was forced by the Nazi government to refuse it, as Hitler had deemed it “un-German”. When Domagk made an attempt to accept the award, he was arrested by the Gestapo and was coerced into writing a letter of refusal to the Nobel committee [3]. Domagk was finally able to accept his Nobel Prize in 1947 [3].
Virtually everyone is acquainted with the antibiotic properties of penicillin, the most famous of all petri-dish molds [3]. In 1929, Sir Alexander Fleming was disposing of old petri dishes from a previous experiment containing the common infectious bacteria, Staphylococci. One dish was contaminated with a mold, Penicillium notatum, and to Fleming’s surprise, he noticed that the bacteria surrounding the mold were dead. Fleming deduced that the mold must have released a substance that killed the bacteria [3].
Due to his lack of expertise in the field of chemistry, Fleming was unable to isolate the antibiotic substance but called the active compound penicillin [3]. With crude penicillin extracts, Fleming showed that it was not toxic to animals and that it selectively killed bacteria [3]. Although the crude product was good enough for the purposes of Fleming’s research, it was not strong enough to fight a serious human infection, making it clinically useless at the time [3]. Fleming presented his results to a Medical Research Club and published his results in the British Journal of Experimental Pathology in 1929; but this failed to create any excitement in the scientific community [3]. The incredible effectiveness of penicillin was not recognized for another ten years.
In 1939, accomplished biochemists Ernst Chain and Sir Howard Florey decided to study antimicrobial substances produced by microorganisms and took on the task of isolating Fleming’s penicillin [3]. Apparently penicillin is not highly concentrated in the mold Penicillium notatum and they discovered that it took gallons and gallons of mold to produce enough penicillin to even cover a human fingernail [3]. Animal trials on the compound began in 1940 and were extremely successful. In 1941, they attempted to treat a human patient: a policeman suffering from a severe bacterial infection. He improved remarkably but eventually worsened and died when the scant supply of penicillin ran out. Losing a patient due to such an arbitrary circumstance upset Florey and he vowed that he would always have enough penicillin on hand to treat an infection completely [3]. He increased the production of penicillin by recruiting 35 British and American organizations ranging from government agencies to pharmaceutical companies [3]. By D-Day of World War II, there was enough penicillin to care for every soldier in need of antibiotic treatment [3]. Penicillin was the only lifesaver of the war and brought many soldiers home that would have otherwise succumbed to bacterial infections contracted from the horrific conditions of military trenches. Following the enormous success of penicillin during wartime, Fleming, Florey and Chain received the Nobel Prize in Physiology and Medicine for the ‘discovery of penicillin and its curative effect in various infectious diseases’ [3].
From this point onward, the use of antibiotics had a huge positive impact on human health. Pneumonia, syphilis, gonorrhea, diphtheria, scarlet fever, wound and childbirth infections that once killed suddenly became treatable and deaths due to infectious disease decreased dramatically [3]. Henry Sigerist, an American medical historian and writer, claimed “most of the infectious diseases have now yielded up their secrets. Many illnesses had been completely exterminated; others had [been brought] largely under control” [2]. The Surgeon General of the United States stated, “It is time to close the book on infectious diseases” [2]. Infectious disease research slowed and medical schools including Yale abandoned it entirely, convinced that it would soon be obselete [2,4]. This optimism prevailed in developed countries as the lethality of infectious disease from generations past was quickly forgotten [2].
The Evolution of Antibiotic Resistance
Fleming recognized the life-saving potential of his penicillin but was also wary of its weaknesses [3]. As early as 1940, rumours of new antibiotic-resistant bacteria began to circulate. A letter to the scientific journal, Nature, was published outlining the discovery of a bacterial enzyme capable of destroying penicillin [3]. In 1946, Fleming himself warned the scientific community: “The administration of too small doses leads to the production of resistant strains of bacteria” [3]. These cautionary words have turned out to be all too true. Bacteria have developed tough and devastating resistance mechanisms that lower the effectiveness of its corresponding antibiotic only a few years after clinical release of the drug(see Table 1) [5]. For example, in the 1940s most gram-positive bacteria were susceptible to Fleming’s penicillin. However, resistance to penicillin was observed as early on as 1946 and in today’s hospitals bacteria such as Staphylococcus are now penicillin-resistant [5]. Infections that at one time were successfully treated with small doses of antibiotics now require large concentrations. More powerful drugs have been synthesized but already resistant bacteria are evolving (Table 1) [5]. For example, 50% of bacteria are resistant to the new antibiotic methicillin and vancomycin, one of the only treatments for methicillin-resistant bacteria, is already failing [5]
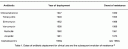
The Mechanisms of Resistant Bacteria
Resistance mechanisms occur as a result of gene mutations: point mutations, deletions, inversions, etc [6]. For each type of antibiotic, a number of different resistance mechanisms may evolve. Currently there are four main mechanisms (see Attack of the Superbugs Figure 2 for a diagram) [6]. First, the bacteria may decrease its uptake of the drug or become completely impermeable to it. This can occur because of a mutated porin [6]. Secondly, the bacteria may accelerate efflux pumps so to increase the export of the antibiotic. In this way, the drug is not in the cell long enough to have a deleterious effect [6]. Bacteria may also develop a way to destroy or enzymatically modify the antibiotic so it can no longer act on its target [6]. Finally, bacteria may inactivate or modify the target of the drug leaving the antibiotic useless [6]. With the evolution of these mechanisms, bacteria can survive antibiotic selective pressures and pass their traits to their offspring.
Bacteria not only transfer antibiotic resistant genes to the next generation but are also able to transfer DNA between bacterial species. This horizontal gene transfer can occur through three mechanisms: transduction, transformation and conjugation (see Attack of the Superbugs:figure 1 for a diagram) [7]. Transduction involves the transfer of DNA from cell to cell by means of viruses. Host DNA that contains a resistance gene may be integrated into the DNA of a mature virus particle, replacing part of the viral genome. When the virus infects another bacteria, the resistant gene is transferred and the recipient bacteria can take on the resistant traits [7]. The second mechanism, transformation, is the process where free DNA from dead bacteria is taken-up and incorporated into the recipient bacteria’s DNA causing genetic change [7]. A third means of resistant gene transfer, conjugation, involves actual cell-cell contact between bacteria. A donor bacterium that has a plasmid carrying resistance genes makes contact with a recipient bacterium via a pili. The cells are then drawn together and the donor transfers a copy of the resistance plasmid to the recipient bacteria. The recipient bacteria not only becomes resistant to antibiotics but is now able to transfer the resistance plasmid to other bacteria [7]. Horizontal gene transfer has a large impact on bacterial evolution. One-fifth of a bacteria’s DNA actually comes from foreign sources that have incorporated their DNA via horizontal gene transfer . This allows rapid and widespread emergence of antibiotic resistance among many bacterial species [6].
Factors Leading to Resistance
Microbial resistance to antibiotics is a natural consequence of selective pressures placed on bacteria. However, humans have greatly accelerated the evolution of resistant bacteria by the overuse and misuse of antibiotics in the community, in the hospital and on the farm [3,5].
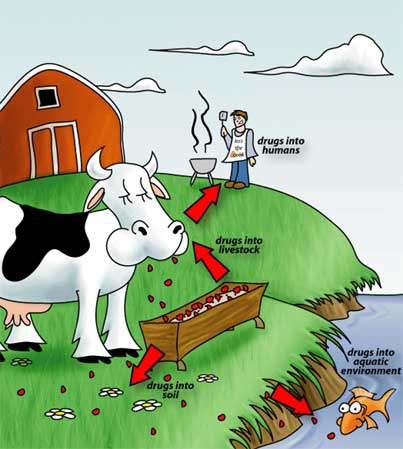
Resistant strains of bacteria are commonly found on farms and have been found in soils and groundwater affected by farm effluents [5]. The abundance of resistant strains in these areas is attributed to the heavy use of antibiotics as growth promoters and therapeutics. In the 1950s, it was noted that antibiotics fed to livestock increased growth rates and animal size leading and thus increased production. It quickly became common practice to include antibiotics in animal feed. When antibiotics began to be used as food additives, there was no regulation behind it. Any antibiotic including those used for human therapy could be used [3].
At the same time, it became common practice to house livestock in confined and concentrated quarters. Farm animals such as chickens when allowed to roam free have limited egg and meat production, so farmers began to collect large numbers of chickens together to increase production. Factory farming led to the rapid spread of infections throughout farms and the use of antibiotics vastly increased to try to counter it [3]. With the common use of antibiotics in farming, resistant genes were emerging in livestock bacteria and residual antibiotics were being ingested by humans, contributing to antibiotic resistance in human pathogens [3].
Fish farming has also contributed to the spread of antibiotic resistance [3]. It is common to put upwards of 100,000 fish into each pen, which creates cramped and stressful conditions. This increases the susceptibility of fish to diseases such as furunculosis. Furunculosis, an infection of the kidneys, is easily transmitted though water and so is devastating to fish farms [3]. To combat the disease, antibiotics were added to fish food. However, the food pellets were unpalatable to the fish who were already suffering from loss of appetite due to their infections. Most of the antibiotics ended up at the bottom of the pens allowing residual drugs to spread throughout the marine environment [3]. Today, vaccines are replacing the antibiotic pellets as a treatment for the disease, but unfortunately most of the damage has already been done [3].
Misuse of antibiotics by humans is another large contributor to the selection of antibiotic resistant bacteria [3,9]. In developing countries, antibiotic prescriptions are often broken up. For example, in India, it is common for pharmacies to sell as many tablets as the patient can afford, which may only be two or three. This is an insufficient dose to cure infection and will only further contribute to the evolution of resistant strains of bacteria [3]. Even when proper antibiotic prescription and use is followed, inadequate dosing still occurs. Recent reports have shown that containers of medicine from Nigeria sometimes contain only half the drug content that was indicated on the label [9]. This may be due to “counterfeit” medicines or improper storage [9]. Tropical conditions such as those in Nigeria readily cause degradation of medical compounds. Although local regulations require pharmacies to store drugs in air-conditioned premises, most undergo frequent power outages and warehouses are rarely kept cool. Furthermore, unauthorized dealers hardly ever bother to follow official storage guidelines [9].
In developed countries, over-prescribing of antibiotics is the main obstacle in slowing the evolution of resistant strains of bacteria [9,10]. In 1998, it was estimated that over-use ranged from 20% to as high as 50% of the community using prescription drugs [10]. With the progressively more hectic lifestyles of both doctor and patients comes the pressure to prescribe. Patients have little time for illness and require an easy cure. Doctors are overworked and have incomes proportional to how frequently they treat individuals and so they have less time to spend with each patient. This leads to increased likelihood of inappropriate prescription of antibiotics [9]. Furthermore, the demands of the patient for a desired treatment is also a problem. For example, if a patient was prescribed antibiotics for a sore throat in the past, they will be more likely to demand that all future sore throats be treated in the same way regardless of the fact that it is not the appropriate treatment [9]. In addition, some countries such as Spain, do not restrict the availability of antibiotics. Pharmacies sell antibiotics over-the-counter, allowing patients to use antibiotics as they see fit, regardless of whether they actually have a bacterial infection or not [3].
Not only is there an increase in antimicrobial resistance but there is a huge decline in the development of new antimicrobial agents. The drugs that attack easy target mechanisms in bacterial cells have already been discovered and in fact no wholly new antibiotics have been identified since the 1960s [11,12]. Cost is a major issue as the expenses of bringing a new drug to market is continually increasing. In 1991, the development of a drug from research to market cost approximately $231 million dollars. In 2003, the cost had increased to over $800 million dollars [12]. This is an extremely high price to pay to develop new drugs and many small companies simply cannot afford it [12]. On the other hand, big pharmaceutical companies are no longer interested in developing antibiotics [13]. There is no money to be made in short-term drug therapies such as antibiotic treatments, whereas researching treatments for chronic illnesses can eventually lead to far more lucrative markets [13]. Moreover, the few new antibiotic drugs that have been synthesized have had difficulties making it to clinical development [13]. Lastly, drug approval is becoming problematic. To get approval from the FDA, the clinical trials must show superiority to drugs already on the market and this is extremely difficult to demonstrate [13]. Even with the increase in clinical demand, a majority of pharmaceutical companies are exiting the antibiotic market [13].
New Technology
Due to the urgent need for a new class of antibiotics, a minority of research groups and pharmaceutical companies are still seeking out unique strategies and are proving that there are still a few discoveries to made [12,13]. Recently, the clinical development of “nature’s antibiotics”, cationic peptides, has been growing [11]. About 20 years ago, they were identified in the lymph of insects and on the skin of frogs. To date, greater than 600 cationic peptides have been observed in virtually all known plant and animal species and have been proven to be an important component of immunological systems [11]. In mammal tissues, several different peptides per single tissue have been found, each at relatively low concentrations [11]. These peptides have been shown to kill microorganisms directly and may do so through several proposed mechanisms. To cross the outer membrane of bacteria, cationic peptides are thought to use self-promoted uptake. Unfolded cationic peptides associate with the negatively charged bacteria surface. They either neutralize a large patch of the membrane or bind strongly to it, effectively disrupting the membrane and translocating across [11]. Once across the outer membrane, the cationic peptides insert into the cytoplasmic membrane leading to bacterial death through four possible outcomes: cell lysis, pore formation in the cytoplasmic membrane, cytoplasmic membrane dissolution, or by attacking internal targets (see Biomedicine: The New Antimicrobials: Cationic Peptides for a diagram)[11]. There is evidence that not only are the cationic peptides antibacterial, they also have important roles in innate immune system function. This includes the chemoattraction of monocytes and neutrophils to the site of infection, stimulation of wound healing, neutralization of endotoxins released by bacteria, inhibition of proteases limiting tissue injury and the stimulation of mast cells to increase vascular permeability allowing infiltration of immune cells into injured tissue [11]. Therefore, cationic peptides have potential as a new class of antibiotics with bactericidal and immune boosting properties.
Cationic peptides have recently been introduced into clinical trials. Micrologix Biotech Inc., a small pharmaceutical company, has synthetically produced and altered the peptide indolicidin, naturally found in bovine neutrophils, to target bacterial infections. Specifically, Micrologix developed a topical cream for acute acne and a coating for central venous catheters [14]. Both drugs have advanced to Phase III trials; however, they are not without problems. They appear to be very toxic in synthetic form and are expensive to make. More work still needs to be done before Micrologix attains successful FDA approval.
Cationic peptides also have agricultural value. Phytopathogens are a major problem causing billions of dollars in lost revenue annually so an effective means to target these pathogens without affecting agricultural productivity is being investigated [15]. Transgenic potato plants have been developed which contain a chimeric peptide created from silk worms and bees [15]. These plants have increased resistance to infection. Furthermore, the potatoes have not been found to be toxic and have maintained their antibacterial properties for more than a year [15]. These findings in clinical and agricultural testing indicate that cationic peptides appear to have real potential in fighting infectious agents.
Other unique approaches to antibiotic research are being presently being pursued. A French biotechnology company, Entomed Inc., is currently studying insects from equatorial countries to discover more antibiotic agents. To date, 25% of all compounds they have identified possess antimicrobial properties. Currently, a cationic peptide isolated from the tobacco budworm is being tested in pre-clinical trials [16]. A group of American scientists is now researching the antibacterial properties of alligator serum. Alligators often sustain serious wounds due to fighting or collisions with boat propellers, however they manage to resist infection in spite of the fact that they live in marshy areas which harbour a high concentration of infectious microbes [17]. To determine how alligators do this, alligator serum has been isolated and tested against the bacteria, Escherichia coli. Compared to human serum, alligator serum is ten times more effective at lowering bacterial survival rates, and its effectiveness is in turn inhibited by proteases, suggesting the discovery of a new protein serum component with antimicrobial properties [17]. An American company, Shaman Pharmaceuticals, is looking to native healers in developing countries for drugs used in traditional healing and is currently testing the compounds in clinical trials. One such product, an extract from sap of the Croton lechleri tree found in the Amazon, is under evaluation to target diarrhea, a symptom of infection [12,18]. Another American company, Phytera, is examining seaweed for antimicrobial agents. Also, a library of potential therapeutic chemicals is being created, which will produce millions of compounds for further screening [12]. By applying traditional screening techniques to unique compounds and substances, these pharmaceutical companies and research groups may increase their chances of discovering a new class of antibiotics.
The optimism that current antimicrobial chemotherapy will last well into the future is declining as new and re-emerging outbreaks of antimicrobial resistant infections continue to spread. Infectious disease will remain a significant problem that will need to be continually and effectively controlled. Managing this issue will require increasing surveillance, establishing proper use of antibiotics in the community and encouraging antibiotic research not only in small companies but in larger pharmaceutical corporations [2,5] Ignoring the speed of the evolution of resistant pathogens will only lead to expensive and disastrous losses when all traditional means for infectious agent control finally fail.
Glossary
- gram-positive- possessing a single cell wall that does not contain
- lipopolysaccharide; these bacteria are identified when they stain violet when rinsed by Gram’s Method
- point mutation- a random change of a single nucleotide pair in a DNA sequence
- deletions- loss of one or more nucleotide base pairs in a DNA sequence
- inversions- a mutation in which a segment of a DNA segment is reversed
- porin- a cell membrane pore protein that does not involve active transport
- efflux pumps- a cell membrane protein that actively transports matter out of the cell
- plasmid- distinct bacterial DNA molecules, not necessary for cell survival, which replicate independently
- pili- a protein filament originating from the cytoplasmic membrane in bacteria which are used for either adhesion or conjugation (the horizontal transfer of DNA from one bacteria to another)
- monocytes- a large, phagocytic white blood cell
- neutrophils- the largest, most physiologically abundant white blood cell
- endotoxins- lipopolysaccharide molecules found on the cell surface of gram-negative bacteria, which is potentially toxic to host cells
- proteases- enzymes which break down protein molecules
- mast cells- cells, that produce histamine, and are involved in the allergic response.
- central venous catheters- a medical device used to drip fluids intravenously into a patient’s central veins
- phytopathogens- infectious agents which invade plant organisms
- transgenic- possessing genes from another species
- serum- the non-cellular component of blood
References
1. McDermott,W. & Rogers,D.E. Social ramifications of control of microbial disease. The Johns Hopkins Medical Journal 151, 302-312 (1982).
2. Cohen,M.L. Changing patterns of infectious disease. Nature 406, 762-767 (2000).
3. Amyes,S.G.B. Magic Bullets Lost Horizons: The rise and fall of antibiotics. Taylor & Francis Inc., London (2001).
4. Janeway,C.A. The Road less Traveled by: The Role of Innate Immunity in the Adaptive Immune Response. The Journal of Immunology 539-544 (1998).
5. Palumbi,S.R. Humans as the world’s greatest evolutionary force. Science 293, 1786-1790 (2001).
6. Normark,B.H. & Normark,S. Evolution and spread of antibiotic resistance. Journal of Internal Medicine 252, 91-106 (2002).
7. Madigan,M.T., Martinko,J.M. & Parker,J. Brock Biology of Microorganisms. Simon & Schuster, New Jersey (1997).
8. Resisting Antibiotics. resistingantibiotics.org 2004. 2-9-2003.
9. Knobler, S. 2003. The Resistance Phenomenon in Microbes and Infectious Disease Vectors. National Academies Press, Washington,D.C. (2003).
10. Harrison, P.F., 1998. Lederberg, J. Antimicrobial Resistance: Issues and Options. National Academy Press, Washington,D.C. (1998).
11. Hancock,W. Cationic peptides:effectors in innate immunity and novel antimicrobials. The Lancet 1, 156-164 (2001).
12. Moellering Jr,R.C. New approaches to developing antimicrobials for resistant bacteria. Journal of Infection and chemotherapy 9, 8-11 (2003).
13. Projan,S.J. Why is big Pharma getting out of antibacterial drug discovery. Current Opinion in Microbiology 6, 427-430 (2003).
14. Micrologix Biotech Inc. www.mbiotech.com . 2004. 2-9-2004.
15. Osusky,M. et al. Transgenic plants expressing cationic peptide chimeras exhibit broad-spectrum resistance to phytopathogens. Nature Biotechnology 18, 1166 (2000).
16. Entomed. www.entomed.com . 2004. 2-9-2004.
17. Merchant,M.E., Roche,C., Elsey,R.M. & Prudhomme,J. Antibacterial properties of serum from the American alligator. Comparative Biochemistry and Physiology 136, 505-513 (2003).
18. Shaman Pharmaceuticals. www.shaman.com . 2004. 2-9-2004.
(Art by Jen Philpot – note that a higher res version of the image file are available here)